Optimizing Analytical Complexities in Emerging Field-Portable Chemical Detection Instruments for Harsh Environments and Public Health Science - Juniper publishers
Journal of Trends in Technical and Scientific Research
Abstract
Exposure scientists often use gas chromatography-mass
spectrometry (GC-MS) systems for analysis of hazardous chemicals [1-3].
Because GC-MS systems with transmission quadrupole mass spectrometers
transmission quadrupole mass spectrometers (TQMS) historically have
limited portability, small ion trap mass spectrometers (ITMS) have been
manufactured to reduce size and increase ruggedness of GC-MS systems [2,
4-8].
The formation of pseudomolecular ions observed in
ITMS spectra are seen as a drawback, potentially leading to chemical
misidentification [9]. It is proposed here that these spectral
complexities can improve analytical power if the pseudomolecular ions
can be used to differentiate chemical isomers, not possible with TQMS.
Isomers of propyl and butyl sulfide were analyzed using TQMS and ITMS.
TQMS spectra exhibited nearly identical peaks for sulfide isomers.
ITMS yielded complex spectra with protonated
molecules, dimers, and adducts. Differentiation of sulfide isomers we
accomplished via characteristic spectral differences in the
pseudomolecular ions formed during detection. The research shows that
field-portable ITMS systems can differentiate chemical isomers, a
capability that may be important for analysis of toxic industrial
chemicals and chemical warfare agents.
Keywords: Gas Chromatography Mass Spectrometry Harsh Environments Public Health Chemistry
Introduction
In the exposure sciences, a need exists for the
development of high-performance analytical equipment with increased
portability. One way in which manufacturers are continuously working to
meet that need is through advancement of emerging technologies to
improve GC-MS chemical identification instrumentation, making detection
systems smaller and faster for field use while striving to maintain or
improve equipment analytical performance. One such advancement is the
advent of field-portable (FP) GC-MS instruments that have been and
continue to be used in a variety of settings over the past two decades.
Although there are a number of GC-MS systems that are
considered portable, they vary in size, weight, power requirements,
attachment needs, sample introduction capabilities, and ease of use.
Some field portable GC-MS (FP-GC-MS) systems are vehicle-mounted or
field-portable but stationary, while others are truly person-portable,
capable of solitary movement and operation in theoretically every
environment in the field by one or two individuals.
The overall purpose of this study was to assess the
instruments that have emerged as field portable and capable and evaluate
the previously reported chemical reactions that occur in ITMS systems
vs TQMS for analytical capability and optimization rather than potential
problems and weaknesses, with the overall goal being to optimize
methods for analysis of hazardous compounds in deployed, emergency
management, forensic investigation, and public health preparedness
settings. This was achieved through the evaluation of field-portable
GC-MS units for the analysis of common industrial hazardous compounds
with partial similarstructures to hazardous compounds and chemical
warfare agents
(CWA) or their components. For legal, logistical, and safety purposes,
live chemical warfare agents could not be used for this
study.
Hypothesis
Ion/molecule reactions that occur during chemical analysis
using small internal ionization ion trap mass spectrometers
(ITMS) will result in the formation of pseudomolecular ions, and
these psuedomolecular ion byproducts can be used to differentiate
isomers of military and emergency-management relevant
chemicals
Background
Emergency operations and accidental hazardous materials releases
Military, forensic, and emergency response operations are
often unique when compared to the day-to-day operations in the
civilian industrial complex, often using unique equipment and
processes, and occurring in unusual environments in which short
notice, on-the-spot solutions to unexpected operational requirements
are not unheard of. The heavy industrial, unexpected, and
high-hazard culture of the military lends itself to the potential for
unexpected emergency responses to accidental chemical releases
and unanticipated exposures to toxic industrial chemicals [10] as
do first response organizations and forensic investigators.
Over the past two decades, there has been a noticeable increase
in the need to establish effective exposure assessment
programs in harsh environments, including military industrial
processes, deployed settings, emergency response and public
health preparedness programs [10-12], and chemical, biological,
radiological, nuclear, and high-yield explosive (CBRNE) programs.
The ability to effectively analyze environmental matrices within
these settings can be vital in decision-making regarding forward
deployment of military troops, law enforcement agents, or emergency
responders, as well as decisions regarding post-exposure
medical and environmental responses, and selection of personal
protective equipment, among other necessary actions. These situations
increase the need for rapid field-based exposure monitoring
equipment.
The existence of field-deployed high-performance analytical
equipment can potentially provide the same level of analytical
results as a laboratory, but in a matter of minutes, providing deployed
commanders the capability to make near real-time decisions
when they are necessary. Rapid on-site field-based chemical
identification can also improve decision-making that will ensure
increased safety and protection of troops and responders in
high-hazard environments [13]. Gas chromatography-mass spectrometry
(GC-MS) is a technology that has proven to be well-suited
for identification of dangerous organic chemicals, including
toxic industrial compounds (TIC), toxic industrial materials (TIM),
and chemical warfare agents (CWA).
The coupling of GC and MS into a hyphenated method (GC-MS)
for chemical identification provides orthogonality, and thus definitive
analytical information for many types of samples including
chemical unknowns within mixtures, with a high degree of certainty
[1,14]. Effective analysis of chemical compounds has been
accomplished with GC-MS systems utilizing transmission quadrupole
(TQMS) and ion trap (ITMS) mass spectrometers [15,16].
The use of laboratory grade GC-MS systems for field applications
is constrained due to the lack of ease of transportability. Numerous
advancements have been made in the development of deployable
GC-MS systems. Nevertheless, the necessity and the ability
to transport, transfer, and effectively use analytical equipment in
the field has been challenging due to the limitations of size, speed,
power, and ruggedness.
There are numerous analytical instruments currently being
used by military, and other non-traditional workers, for chemical
identification, including a number of portable GC instruments
which have been shown to be well-suited for the identification and
quantification of expected analytes. Field-portable (FP) GC-MS
systems have been developed and applied in many settings including
forensic investigations of explosives, fires, airborne toxicants
in war zones, natural disasters, and environmental assessments
[1,12, 17]. Nevertheless, the early systems suffered from incapability
to perform liquid injections, poor chromatography related to
the GC-MS membrane interfaces, inability to analyze compounds
with relatively low volatility, inadequate battery life, slow analysis,
and high maintenance requirements [1,13]. As a result of increased
market demand for truly field-portable systems, considerable
effort has gone into improving capabilities of field-portable
GC-MS instruments, including portability and performance [4].
Well-known advances include the use of low thermal mass (LTM)
resistively heated GC capillary column assemblies which significantly
increase sample throughput and speed while simultaneously
reducing the overall instrument size [2,18].
The small LTM GC assembly is capable of rapid analysis with
high chromatographic performance, and when combined with
small MS detectors, further instrument size and power reductions
are realized compared to earlier systems [2-5,7-9]. As such, a
need exists for continuous improvement of FP GC-MS instrument
options, and smaller systems utilizing miniature ITMS detectors
have been developed to meet this need. In addition to the claim
that ITMS analyzers are 10-100 times more sensitive than their
ion beam quadrupole cousins, ITMS technology has allowed advances
in mass analyzer miniaturization [4,5,8,9,19], which has
been touted as the biggest advancement for field use by military,
environmental, and emergency preparedness users.
Internal ionization ITMS instruments are ideal
candidates for
miniaturization because they are inherently small and contain few
or no ion optic elements, and thus do not require highly precise
alignment. Additionally, ITMS detectors can operate at higher
pressure than other MS types. This occurs because helium (He)
buffer gas has an effect of collisionally dampening ion motion
tocollapse ions to the center of the ion trap [20]. Since the field is
more homogenous in the center of the trap, the ions stored closer
to the center of the trap will experience a more uniform ejection
process, leading to improved mass spectrometer performance
[20]. This ability to operate at higher pressure eases requirements
for the pumping systems, thus allowing for smaller pumps that
use less power [5,20].
Furthermore, the amplitude of the applied radio frequency
(rf) trapping potential is inversely proportional to the square of
the analyzer radial dimension. Therefore, a decrease in analyzer
size results in a reduction of operating voltage, hence, lower power
requirements [5]. Thus, miniaturization of ITMS detectors with
smaller GC systems has been explored for best potential FP GC-MS
design.
Although technological advances have been made, for over a
decade, chemists and other experts have reported that pseudomolecular
ion formation in ITMS systems as a result of in-trap
chemical reactions for certain analytes, observed in spectra as
fragment/neutral adducts, protonated monomers, and dimers,
are problematic for analysis by technicians and analysts in the
field and may lead to chemical misidentification [9]. Though these
pseudomolecular ions have been viewed as an analytical complication,
it is possible that their complex spectral data can be explored
for unique identification purposes.
A few manufacturers have focused on developing overall combined
instrument miniaturization by coupling the smaller and
faster LTM GC with miniaturized ITMS analyzers. A miniature
cylindrical ion trap (CIT) mass analyzer was developed and coupled
with LTM GC to form the Griffin Model 450 GC-CITMS system
(ICX-Griffin, West Lafayette, IN), which has dimensions (48.8 cm x
48.8 cm x 53.6 cm) and weight (43.5 kg) considerably smaller and
lighter than laboratory or vehicle mounted transmission quadrupole
GC-MS systems [5] (Figure 1a).
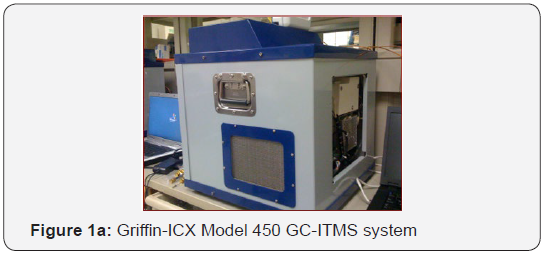
These small internal ionization ion trap GC-MS systems have
the advantage of size, weight, and reduced power requirements, as
well as improved sensitivity, lending to better overall portability
and performance in deployed settings [1,2]. Small ITMS instruments
also possess analytical capabilities not present in transmission
quadrupole EI/MS detectors. The ion/molecule interactions
that may be observed in internal ionization ITMS mass spectra exemplify
self-chemical ionization (self-CI) leading to self-ion-molecule
reactions (SIMR), and the formation of pseudomolecular ionscommonly observed in mass spectra for certain analytes as fragment/
neutral adducts, protonated molecules, and dimers [1,21-
24].
Although these SIMR reactions are often viewed as a drawback
for field technicians and field analysts, self-CI in ITMS may
possibly be useful for locating molecular ions in the spectra of
certain classes of compounds, allowing for a definitive identification
or as an additional source of evidence. Formation of characteristic
peaks during self-CI can be useful for the identification of
unknown compounds without the necessity of chemical reagents
required in traditional CI [9,4]. This technique could potentially be
applied to perform SIMR for isomer differentiation [4-8,23]. This
study focused on exploring the possibility of using FP GC- ITMS
systems for isomer differentiation of hazardous and CWA-related
chemicals.
Experimental Methods and Procedures
Objectives
The purpose of this study was to investigate whether SIMRs
that occur in small internal ionization ITMS systems can be used
to differentiate isomers of sulfides commonly seen as components
or byproducts of military-relevant chemicals, such as CWAs.
Materials and Methods
The Griffin Model 450 (Figure 1a) FP GC-ITMS system (Griffin-
ICX Technologies, West Lafayette, IN) was used to analyze the
chemicals of interest. The Griffin FP GC-ITMS is outfitted with a
low thermal mass (LTM) Gas Chromatograph. For this study, it was
equipped with a 30m resistively heated DB-5MS capillary GC column.
Ultra-high purity (UHP) helium was used as a carrier gas for
every experiment. The injector temperature was set at 250 °C, and
the initial column temperature was set to 40°C with a temperature
hold time of 60s.
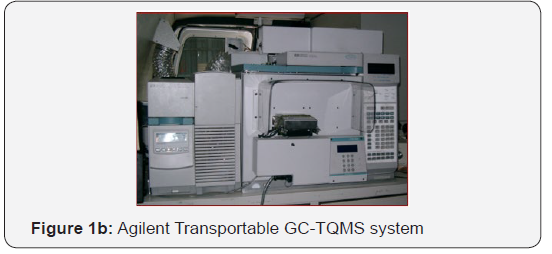
The temperature ramp rate was established at 40°C per minute
to a terminal column temperature of 300°C, and terminal temperature
hold time of 60s. The instrument was operated in splitless
mode, and the mass range was 40 to 425amu. The FP GC-ITMS was
used to obtain mass spectra for propyl and butyl sulfide isomers.
In this study, dipropyl, isopropyl, di-n-butyl, and sec-butyl sulfides
were analyzed. The sulfides were obtained commercially (Aldrich,
Milwaukee, WI). Spectral analysis for the ITMS instrument was
conducted using the manufacturer-designed Griffin Systems Software
(GSS) program (Griffin-ICX Technologies, West Lafayette, IN).
The experiment was repeated using a laboratory-grade Agilent
6890 GC coupled with a 5975 TQMS (Agilent Technologies, Wilmington,
DE). Sulfide sample introduction was performed using solid
phase microextraction for both systems. For both instruments,
the injector temperature, initial column (Figure 1b) temperature,
initial temperature hold, temperature ramp rate, terminal column
temperature, and terminal temperature hold were identical, and
both were operated under split less injection mode.
The small ITMS instrument was compared with the TQMS for
the ability to differentiate sulfide isomers. The mass spectra produced
during the analysis of the introduced sulfide isomers were
examined for formation of pseudomolecular ions as protonated
molecules [M+H] +, adducts [M+F] +, and dimers [2M+H] +. Data
collected using TQMS was analyzed with the Agilent MSD Chem-
Station software (Agilent Technologies, Wilmington, DE). The
National Institute of Standards and Technology Mass Spectral Library
(NIST, Gaithersburg, MD) was used as a reference standard
for spectral analysis.
Results
The formation of [M+H]+ at m/z 119, [M+F]+ at m/z 174, 179,
and 207, and the dimer ions [2M]+ and [2M-H]+ at m/z 235 and
236 respectively, were observed for propyl sulfide (Figure 3a).Only the molecular ion (m/z 118) and [M+H] + (m/z 119) were
observed for isopropyl sulfide (Figure 3b). The results from the
analyses of the butyl sulfides were similar. TQMS analysis for the
butyl sulfide isomers were similar and no pseudomolecular ions
were observed (Figure 4).
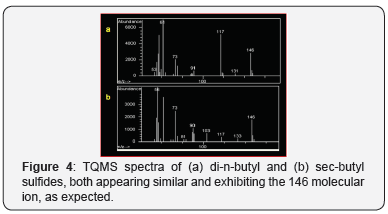
The molecular ion for both was observed at m/z 146 as expected.
ITMS analysis yielded the formation of pseudomolecular
ions for both isomers. For di-n-butyl sulfide, [M+H]+ was observed
at m/z 147, as exhibited in Figure 5a. Adducts were also observed
(m/z 202, 207, and 249) as well as dimer ions [2M+H]+ and [2M]+
(m/z 292 and 293, respectively). The protonated monomers and
adducts [M+H]+ (m/z 147) and [M+F]+ (m/z 201, 207) were observed
when sec-butyl sulfide was analyzed with the ITMS, but no
dimer was observed (Figure 5b).
.png)
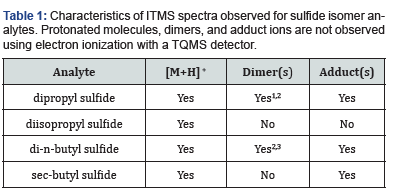
Due to low pressure and rapid transit of ions created in the
ion beam TQMS instrument, ion/molecule reactions do not occur,
and resulting mass spectra are consistent and standardized. In
contrast, the ITMS instrument produced ion current for the pseudomolecular
ions for all four analytes as predicted. The differentiation
results can be seen in Figures 2-5 and Table 1. The resulting
presence/absence of protonated monomers, adducts, and dimerswere used to differentiate the sulfide isomers.
Discussion
Formation of pseudomolecular ions as protonated molecules,
adducts, and dimers may be affected by steric effects in which the
size, space, or arrangement of atoms within the molecule affect
the energies and potential reactions. Additionally, the sizes and
geometries of side chains in the studied analytes can have an effect
on electrophilicity of cations produced by EI, and thus the ability
to react. A branched structure will tend to have more resonance
structures when compared to a straight chain, leading to electrophile
stabilization. Thus, branching tends to result in a decrease
of reactions and a subsequent reduction or elimination of adducts
observed.
This phenomenon was observed during the analysis of the
propyl sulfide and butyl sulfide isomers. The straight-chain isomers
(propyl and dibutyl sulfide) both exhibited the formation of
[M+H]+, multiple adducts, and multiple dimers, compared to the
branched chain isomers, which exhibited fewer pseudomolecular
ions. These resulting differences in adducts and dimers observed
in mass spectra, between isomers, can be used to differentiate
those isomers from one another, potentially allowing for a higher
degree of certainty during routine or emergency response chemical
identification operations.
The resulting presence/absence of protonated monomers, adducts,
and dimers were used to differentiate the sulfide isomers,
showing an advanced capability not possible with larger transportable
systems with TQMS detectors. Therefore, although the
pseudomolecular ion formation that occurs as a result of reactions
in the ion trap may make identification slightly difficult for an inexperienced
or untrained technician or analyst, increased and
improved education and training may not only correct this ability
gap, but may improve the overall capability of field analysts to
identify compounds with a higher degree of certainty as a result of
the capability to differentiate isomers.
Other Considerations
Use of GC-ITMS technologies in combination with modern and emerging computer-based communications technologies
Additionally, despite the technical and scientific gaps that potentially
exist between lab scientists and field technicians and analysts,
the gap may also potentially be closed as a result of emerging
technologies in high-speed internet, bandwidth, cloud computing,
cyber-security, and telepresence communications. Miniaturization,
speed, capability, and power of analytical instruments has
been critical to field-based analysis and near real-time answers
regarding potential exposures. However, advancements in other
technological arenas are also potentially beneficial to emergency
preparedness and response, among other field-based operations.
Numerous advancements have been made in the world of
wireless high-speed internet, bandwidth availability, and cloud
computing. There is the potential that gaps in technician
training,ability, and knowledge can be overcome if data collected by
technicians
in the field can be transported and analyzed in real-time by
subject matter expert scientist analysts located at a distance. This
can be potentially accomplished through the use of existing video
communication technology platforms in combination with secure
E-mail and other streaming technologies, as well as through the
use of emerging communication technologies such as holoportation,
an advanced system that uses mixed reality and heads up displays
to provide near telepresence.
Use of GC-ITMS technology with combination of holoportation communications
Mixed reality technologies combine virtual reality and augmented
reality (VR/AR), and are being studied for and employed
in medicine, education, psychological treatment, architecture,
rehabilitation, and tourism [25,26-31], among other fields. The
advancement of VR/AR devices has increased the way communications
can be used. Mixed reality involves using computer hardware,
software, capture devices, displays, sensor, and trackers for
the effective collection, compression, and dissemination of the aggregated
data [29].
What this does is allow users to insert virtual content into real
world content, permitting both to run simultaneously in real-time
[26]. The technology has already been used repeatedly for inter-
group military participation, including multi-participant battlefield
exercises involving multiple defense agencies, in real-time.
But even these uses fall short compared to the emerging capability
of telepresence, which is the ability of a participant to appear present
at a remote location. When combined, VR/AR allow scenarios
that are nearing complete telepresence through what is called holoportation
[31,32].
Holoportation takes not only distance communication, but
VR/AR to another level, combining these modalities to produce
technology that offers a dynamic, 3-D holographic presence. This
technology is practically an end-to-end system of real-time reconstructions
of space, surroundings, and people, with the ability to
transmit those recreations to remote users, affording immediate,
3-D visual, auditory, and emotional interactions between users at
remote settings [33].
In this technological method, a series of specially outfitted
cameras is situated in the space to capture the participants and
their surroundings three-dimensionally. These cameras capture
the data and the developed software fuses the data to create a
temporally consistent model. These cameras are also outfitted
with devices such as the Hololens™ tracking technology that aid
the cameras in taking the 3-D models and compositing them in
real-time into the real world. The reconstructions are textured,
and the complete data are compressed and transmitted to similar
set-ups on the other end, where the receiving user wearing the
Hololens™ can see the remote participant live, in his or her space,
as though they are co-present in the same location [33,34].
Holoportation allows people who are distances away to havethe experience of being present in the same time and space, offering
numerous advantages. Holoportation can potentially connect
technicians in the field with scientists and other subject matter
experts in the lab or other safe location, even thousands of miles
away, and allow the experts to perform observation and analysis of
the chemical data sampled using the chemical analyzers, providing
an expert and more definitive answer from miles or thousands
of miles away for operational decision-makers in the danger-zone
[31]. Thus, whether it be performed through currently available
2-D platforms in combination with E-mail transmission of data
files, or through the emerging telepresence available through holoportation,
it appears that there is a potential that the challenge of
chemical analysis and the complexities of spectral differentiation
by complex systems may fall not into the hands and minds of field
technicians and scientists, but perhaps in the hands of cyber-security
and cryptology professionals that must ensure data integrity
and security.
Conclusion
Exposure scientists use GC-MS systems for analysis of hazardous
chemicals and materials to prevent military exposures, environmental
exposures, public health issues, and to gather forensic
evidence. Because even the most transportable GC-MS systems
with TQMS detectors historically have limited portability due to
size, power, and ruggedness concerns, small ITMS have been manufactured
to reduce size and increase ruggedness [2,4-8] of combined
GC-MS systems, making them more apt for deployment to
the field by military special operations, environmental health, and
CBRNE experts.
Despite the benefits of significantly smaller instruments due
to the ability to miniaturize GC-ITMS instruments, the complexities
of analysis of ITMS spectra, compared to its TQMS cousin,
have been touted as a potential hindrance to operational effectiveness,
particularly due to the formation of pseudomolecular ions
observed in ITMS spectra. Some analysts and scientific strategists
believe that more complex spectra potentially leading to chemical
misidentification by operators, technicians, and field analysts,
generally requiring a more experienced GC-MS subject matter expert
to ensure accuracy of data [9,4].
However, the chemical reactions that occur in the ion trap and
lead to the formation of the pseudomolecular ions may not be as
much of a drawback as some believe. It was proposed in this study
that these spectra can actually improve analytical power if the
pseudomolecular ions are used to differentiate chemical isomers,
not possible with TQMS. The ability to not only identifying the
chemicals but being able to actually differentiate isomers leads to
an increased level of identification as well as an additional level of
identity confirmation. The researchers analyzed isomers of propyl
and butyl sulfide using GC-TQMS and GC-ITMS instruments and
compared the spectral analyses. TQMS spectra exhibited nearly
identical peaks for sulfide isomers. ITMS yielded complex spectra
with protonated molecules, dimers, and adducts. Differentiation of
the sulfide isomers were accomplished via characteristic spectraldifferences in the pseudomolecular ions formed in the ion trap.
In summary, field-portable FP GC-MS systems with miniature
ITMS detectors can be used to differentiate chemical isomers
through analysis of their self-CI byproducts, a capability that can
be important for analysis of toxic industrial chemicals, chemical
warfare agents, and other compounds important to military,
emergency response, and forensic professionals, among others.
Thus, the self-CI and the formation of pseudomolecular ions previously
viewed as a drawback can actually be used as an attribute of
the smaller detectors. Furthermore, in a time of rapidly advancing
technological emergence, the gaps and weaknesses supposed to
be deficiencies of the use of these systems in field settings may be
void as analytical assessments, processes, and determinations can
be made by remote analysts that are potentially able to analyze
transmitted data in near real-time, or even evaluate the data and
instruct technicians through the use of telepresence technologies,
such as holoportation. Future research should explore quantification
of chemical compounds using pseudomolecular ion data.
Additional research should be conducted regarding the collection,
transmission, distance-analysis, and telepresent analysis of chemical
compounds.
Disclaimer Statement
This project was not funded or sponsored by any particular
institution. It consists of independent research by the authors, and
the authors alone are responsible for the findings and opinions of
the research as presented in the article. Although the authors were
affiliated with numerous institutions during the course of the research
and manuscript development, the findings, interpretations,
opinions, and statements discussed in the article do not represent
any official stance or position of Engineering Global, Nova
Southeastern University, The Uniformed Services University of the
Health Sciences, Naval Hospital Camp Pendleton, The Academy of
Interdisciplinary Health Science Leaders, The Defense Threat Reduction
Agency, the US Navy, or the US Army.
To Know More About Trends in Technical and
ScientificResearch Please click on:
https://juniperpublishers.com/ttsr/index.php
To Know More About Open Access
Journals Please click on:
Comments
Post a Comment